Dawn of clean energy: Enhanced heat transfer, radiative cooling, and firecracker-style controlled nuclear fusion power generation system
DOI:
https://doi.org/10.18686/cest.v1i1.61Keywords:
principle of firecrackers; controlled nuclear fusion; flywheel energy storage; enhanced heat transfer; radiative coolingAbstract
Global climate change has become a major environmental threat and development challenge facing humanity. Controllable nuclear fusion is a globally recognized ideal solution for clean energy, but its required high-energy triggering conditions and intense energy release prevent existing technologies from achieving safe, stable, and long-term continuous operation. Here, inspired by the traditional Chinese firecrackers, we propose a pulsed fusion reaction flywheel energy storage multi-reactor relay operation to drive the steam turbine to continuously and stably generate electricity for a long period of time; meanwhile, to install cleaning rotors in the cooling medium pipeline to enhance heat exchange, and to apply radiative cooling technology on the surface of the cooling tower to improve cooling efficiency and to reduce energy consumption, thereby improving system safety and overall energy efficiency. Proposing the combination of original technologies at both the hot end and the cold end of the system, we strive to open up a new way for controllable nuclear fusion power generation.
References
1. Guo L, Ou Z, Liu Y, et al. Technological innovations on direct carbon mitigation by ordered energy conversion and full resource utilization. Carbon Neutrality 2022; 1: 4. doi: 10.1007/s43979-022-00009-5
2. Wang N. Fusion Energy and Its Future (Chinese). Tsinghua University Press; 2001.
3. Lindl J. Development of the indirect-drive approach to inertial confinement fusion and the target physics basis for ignition and gain. Physics of Plasmas 1995; 2: 3933–4024. doi: 10.1063/1.871025
4. Fisch NJ. Plasma physics and controlled nuclear fusion. In: Libby SB, van Bibber KA (editors). Modern Physics and the Scientific Legacy of Edward Teller, Proceedings of the Edward Teller Centennial Symposium; 28 May 2008; California, USA. World Scientific; 2010.
5. Wesson J, Campbell DJ. Tokamaks. Oxford University Press; 2011.
6. Barabaschi P, Kamada Y, Shirai H, JT-60SA Integrated Project Team. Progress of the JT-60SA project. Nuclear Fusion 2019; 59(11): 112005. doi: 10.1088/1741-4326/ab03f6
7. Zhuang G, Li GQ, Li J, et al. Progress of the CFETR design. Nuclear Fusion 2019; 59(11): 112010. doi: 10.1088/1741-4326/ab0e27
8. Li J, Wan Y. Present state of Chinese magnetic fusion development and future plans. Journal of Fusion Energy 2019; 38: 113–124. doi: 10.1007/s10894-018-0165-2
9. Ye H. A brief analysis of EAST tokamak and related fusion research in China (Chinese). Science and Innovation 2021; 12: 92–93. doi: 10.15913/j.cnki.kjycx.2021.12.037
10. Pfalzner S. An Introduction to Inertial Confinement Fusion. CRC Press; 2006.
11. Zhao Y, Xiao G, Li F. Current status and development of inertial confinement fusion physics research based on modern gas pedals (Chinese). Physics 2016; 45(2): 98–107. doi: 10.7693/wl20160204
12. Basov NG, Ivanov YS, Krokhin ON. Neutron generation in spherical irradiation of a target by high-power laser radiation. Journal of Experimental and Theoretical Physics Letters 1972; 15(10): 417–419.
13. Pavlovskiĭ AI. Reminiscences of different years. Soviet Physics Uspekhi 1991; 34(5): 429. doi: 10.1070/PU1991v034n05ABEH002378
14. Gao S, Wu X, He Z, et al. Research progress of fabrication techniques for laser inertial confinement fusion target. High Power and Particle Beams 2020; 32: 032001. doi: 10.11884/HPLPB202032.200039
15. Huang H, Ma L. Current status of fusion energy development (Chinese). China Science and Technology Information 2023(4): 120–122, 125.
16. Yang W, Zhang E. Pulsed Microfluidic Fusion Impulse Flywheel Control Continuous Power Generation Technology (Chinese). CN Patent 116,110,617, 12 May 2023.
17. Yang W, Zhang E. Microfluidic Deuterium-Tritium Collision Nuclear Fusion Flywheel Control Continuous Power Generation Technology (Chinese). CN Patent 116,434,980, 14 July 2023.
18. Stacey WM. Fusion Plasma Physics, 2nd ed. Wiley-VCH; 2013.
19. Lyublinski IE, Vertkov AV. Experience and technical issues of liquid lithium application as plasma facing material in tokamaks. Fusion Engineering and Design 2010; 85(6): 924–929. doi: 10.1016/j.fusengdes.2010.08.036
20. Humrickhouse PW, Merrill BJ, Yoon SJ, Cadwallader LC. The impacts of liquid metal plasma-facing components on fusion reactor safety and tritium management. Fusion Science and Technology 2019; 75(8): 973–1001. doi: 10.1080/15361055.2019.1658464
21. Bergles AE. Advanced enhancement-third generation heat transfer technology or “the final frontier”. Transaction of the Institute of Chemistry Engineering 2001; 79 (Part A): 437–444.
22. Webb RL, Eckert ERG. Application of rough surfaces to heat exchanger design. International Journal of Heat and Mass Transfer 1972; 15(9): 1647–1658. doi: 10.1016/0017-9310(72)90095-6
23. Zimparov V. Extended performance evaluation criteria for enhanced heat transfer surfaces: Heat transfer through ducts with constant heat flux. International Journal of Heat and Mass Transfer 2001; 44(1): 169–180. doi: 10.1016/S0017-9310(00)00074-0
24. Wang S, Li ZX, Guo ZY. Novel concept and device of heat transfer augmentation. In: Proceedings of 11th International Conference of Heat transfer; 1 November 1998; Kyongju, Korea. Taylor Fancis; 1998. pp. 405–408.
25. Guo ZY, Li DY, Wang BX. A novel concept for convective heat transfer enhancement. International Journal of Heat and Mass Transfer 1998; 41(14): 2221–2225. doi: 10.1016/S0017-9310(97)00272-X
26. Guo Z. Physical mechanism of convective heat transfer and its control: Synergy between velocity and heat flow fields (Chinese). Science Bulletin 2000; 45(19): 2118–2122. doi: 10.3321/j.issn:0023-074X.2000.19.020
27. Guo Z, Wang S. Novel concept and approaches of heat transfer enhancement. In: Proceedings of Symposium on Energy Engineering in the 21st Century (SEE2000) Volume I–IV. Begell House; 2002. pp. 118–126. doi: 10.1615/SEE2000.90
28. Tao W, He Y, Huang P. Numerical validation of field synergy theory in an alternating flow slit-type heat rector (Chinese). Journal of Engineering Thermophysics 2003; 24(4): 649–651.
29. He Y, Lei Y, Tian L, et al. Exploration of the three-field synergy of high-efficiency low-resistance enhanced heat transfer technology (Chinese). Journal of Engineering Thermophysics 2009; 30(11): 1904–1906.
30. Xia X, Zhao L, Xu H, Yang S. Comprehensive performance evaluation factors for enhanced heat transfer based on field synergy theory (Chinese). Thermodynamic Engineering 2011; 26(2): 197–201.
31. Zhang Z. Research on Heat Transfer Enhancement Mechanism and Performance of Assembled Rotors (Chinese) [PhD thesis]. Beijing University of Chemical Technology; 2014.
32. Li Y, Ding Y, Yang W. Numerical analysis of the working principle of cleaning rotors and its industrial application (Chinese). Petrochemical Equipment 2009; 38(6):73–76.
33. Yang W, Li F, Chen S, Yan H. Industrial experimental study of combined pipe-end rotor enhanced heat transfer device (Chinese). Thermodynamic Engineering 2008; 23(4): 378–381.
34. Li FX, Ding YM, Guan CF, et al. Laboratory investigation and commercial test for rotors-assembled strand applied in smooth tube. Experimental Thermal and Fluid Science 2008; 33(1): 1–9. doi: 10.1016/j.expthermflusci.2008.04.005
35. Cui Y, Luo X, Zhang F, et al. Progress of passive daytime radiative cooling technologies towards commercial applications. Particuology 2022; 67: 57–67. doi: 10.1016/J.PARTIC.2021.10.004
36. Li T, Zhai Y, He S, et al. A radiative cooling structural material. Science 2019; 364(6442): 760–763. doi: 10.1126/science.aau9101
37. Yin X, Yang R, Tan G, Fan S. Terrestrial radiative cooling: Using the cold universe as a renewable and sustainable energy source. Science 2020; 370(6518): 786–791. doi: 10.1126/science.abb0971
38. Wang H, Xue C, Guo X, et al. Superhydrophobic porous film for daytime radiative cooling. Applied Materials Today 2021; 24: 101100. doi:10.1016/J.APMT.2021.101100
39. Zhong H, Li Y, Zhang P, et al. Hierarchically hollow microfibers as a scalable and effective thermal insulating cooler for buildings. ACS Nano 2021; 15(6): 10076–10083. doi: 10.1021/ACSNANO.1C01814
40. Liang J, Wu J, Guo J, et al. Radiative cooling for passive thermal management towards sustainable carbon neutrality. National Science Review 2023: 10(1): nwac208. doi: 10.1093/NSR/NWAC208
41. Poredoš P, Wang R. Sustainable cooling with water generation. Science 2023; 380(6644): 458–459. doi: 10.1126/science.add1795
42. Cai C, Chen W, Wei Z, et al. Bioinspired “aerogel grating” with metasurfaces for durable daytime radiative cooling for year-round energy savings. Nano Energy 2023; 114: 108625. doi: 10.1016/J.NANOEN.2023.108625
43. Li J, Fu Y, Zhou J, et al. Ultrathin, soft, radiative cooling interfaces for advanced thermal management in skin electronics. Science Advances 2023; 9(14): eadg1837. doi: 10.1126/sciadv.adg1837
44. Fixen DJ. The temperature of the cosmic microwave background. The Astrophysical Journal 2009; 707(2): 916–920. doi: 10.1088/0004-637X/707/2/916
45. Hossain MM, Gu M. Radiative cooling: Principles, progress, and potentials. Advanced Science 2016; 3(7): 1500360. doi: 10.1002/advs.201500360
46. Zhao D, Aili A; Zhai Y, et al. Radiative sky cooling: Fundamental principles, materials, and applications. Applied Physics Reviews 2019; 6(2): 021306. doi: 10.1063/1.5087281
47. Farooq AS, Zhang P, Gao Y, Gulfam R. Emerging radiative materials and prospective applications of radiative sky cooling—A review. Renewable and Sustainable Energy Reviews 2021; 144: 110910. doi: 10.1016/J.RSER.2021.110910
48. Raman AP, Anoma MA, Zhu L, et al. Passive radiative cooling below ambient air temperature under direct sunlight. Nature 2014; 515(7528): 540–544. doi: 10.1038/nature13883
49. Zhai Y, Ma Y, David SN, et al. Scalable-manufactured randomized glass-polymer hybrid metamaterial for daytime radiative cooling. Science 2017; 355(6329): 1062–1066. doi: 10.1126/science.aai7899
50. Tian Y, Liu X, Wang Z, et al. Subambient daytime cooling enabled by hierarchically architected all-inorganic metapaper with enhanced thermal dissipation. Nano Energy 2022; 96: 107085. doi: 10.1016/J.NANOEN.2022.107085
51. Chen Y, Dang B, Fu J, et al. Cellulose-based hybrid structural material for radiative cooling. Nano Letters 2021; 21(1): 397–404. doi: 10.1021/ACS.NANOLETT.0C03738
52. Son S, Jeon S, Chae D, et al. Colored emitters with silica-embedded perovskite nanocrystals for efficient daytime radiative cooling. Nano Energy 2020; 79: 105461. doi: 10.1016/j.nanoen.2020.105461
53. Li X, Peoples J, Yao P, Ruan X. Ultrawhite BaSO4 paints and films for remarkable daytime subambient radiative cooling. ACS Applied Materials & Interfaces 2021; 13(18): 21733–21739. doi: 10.1021/ACSAMI.1C02368
54. Gao H, Zhang F, Tang K, et al. Green cleaning of 3D-printed polymeric products by micro-/nano-bubbles. Nanomaterials 2023; 13(11): 1804. doi: 10.3390/NANO13111804
55. Zhou L, Li Z. Exploring the standard system of micro bubble technology. Water Purification Technology 2021; 40: 75–87.
56. Zhang L, Chen H, Li Z. Long lifetime of nanobubbles due to high inner density. Science in China Series G: Physics Mechanics & Astronomy 2008; 51(2): 219–224. doi: 10.1007/s11433-008-0026-5
57. Yu Z, Li J, Zhang X. A new hypothesis for cavitation nucleation in gas saturated solutions: Clustering of gas molecules lowers significantly the surface tension. Chinese Journal of Chemical Engineering 2022; 50: 347–351. doi: 10.1016/J.CJCHE.2022.06.009
58. Li P, Wang J, Liao Z. Microbubbles for effective cleaning of metal surfaces without chemical agents. Langmuir 2022; 38(2): 769–776. doi: 10.1021/ACS.LANGMUIR.1C02769
59. Zhang R, Gao Y, Chen L, et al. Nanobubble boundary layer thickness quantified by solvent relaxation NMR. Journal of Colloid and Interface Science 2022; 609: 637–644. doi: 10.1016/J.JCIS.2021.11.072
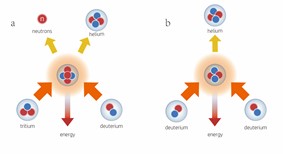
Downloads
Published
How to Cite
Issue
Section
License
Copyright (c) 2023 Weimin Yang, Enxiang Zhang, Jiuzhou Zhao, Yifan Zhao, Kangkang Tang, Yan Cui, Xianyu Luo, Zhen Zhang, Chengjun Li, Fenghua Zhang, Xiaodong Gao

This work is licensed under a Creative Commons Attribution-NonCommercial 4.0 International License.